Progress in Pediatric Cardiology 56 (2020) 101198

Red blood cell mechanical fragility as potential metric for assessing blood damage caused by implantable durable ventricular assist devices: Comparison of two types of centrifugal flow left ventricular assist devices
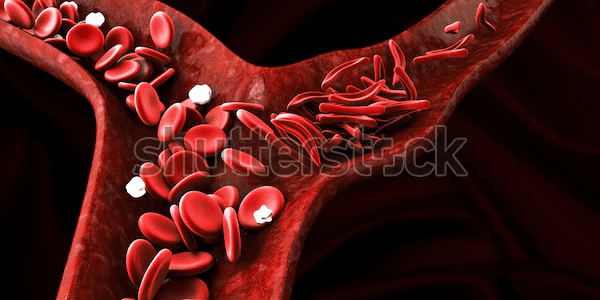
M. Taraseva,b,⁎, S. Chakrabortya, L. Lightc, K. Alfanoa,d, F.D. Paganid
a Blaze Medical Devices, 540 Avis Dr., Ste. A, Ann Arbor, MI 48104, USA
b Functional Fluidics, 440 Burroughs St., Ste. 641, Detroit, MI 48202, USA
c Ontario HIV Treatment Network, 1300 Yonge Street, Ste. 600, Toronto, Ontario M4T 1X3, Canada
d Department of Cardiac Surgery, University of Michigan, 500 S. State St., Ann Arbor, MI 48109, USA
A B S T R A C T
Implantable Ventricular Assist Devices (VADs) have become a treatment of choice for patients with end-stage heart failure or cardiogenic shock, significantly increasing both survival rates and the quality of life of patients. Moreover, VAD use is growing as destination therapy for patients who require permanent mechanical cardiac circulatory support. This heightens the need to ensure VAD reliability and safety, even amidst challenges in optimization of pump design for minimal blood damage. Advanced design LVADs like the HVAD (Medtronic, Inc., Minneapolis, MN) and the Heartmate 3 (HM3; Abbott Labs, Chicago, IL) are centrifugal systems that had been favorably compared to the continuous flow axial pumps, such as the HeartMate II (HMII; Abbott Labs, Chicago, IL). While the HVAD and HMII are increasingly utilized in older pediatric populations in addition to the Berlin Heart Excor (Berlin Heart GmbH, Berlin Germany), implementation of VAD support for pediatric patients still lags behind adults. Recently, the HM3 has demonstrated superior performance to the HMII with respect to pump thrombosis in clinical trials and may also find utility in pediatric applications, particularly for adolescents. In this present work, performance of HVAD and HM3 were compared using basic laboratory tests and Red Blood Cell (RBC) Mechanical Fragility (MF), an assay that provides assessment of sub-hemolytic RBC damage which can be caused by VAD operation. RBC MF was assessed using electromagnetically driven bead milling with cylindrical beads in multiple different regimes for different sample stressing configurations. Induced hemolysis in the sample was measured non-invasively at different (increasing) cumulative stress duration intervals, to obtain a MF profile for each stressing regime. In a cohort of 13 HVAD and 7 HM3 patients, blood samples were obtained before surgery and at 1 h, 24 h, 1 week and 4 weeks after surgery. No significant differences were observed between the two VAD devices in conventional hemolysis markers including free hemoglobin, bilirubin, total LDH and hap- toglobin, as well as in changes patient hemoglobin. HM3 demonstrated elevated, compared to HVAD, levels of LDH-1 at 24 h (p < 0.05) and 1 week (0.05 < p < 0.1) after surgery, with LDH-1 reverting to about pre-surgery levels at 4 weeks. These differences between pumps may have been attributed to confounders such as duration of cardiopulmonary bypass at time of LVAD implant. By one metric, RBC MF was similarly elevated for HM3 at both 24 h and 1 week after surgery, and also reverted to about pre-surgery levels at 4 weeks. Such changes in RBC MF results were observed for only one of the employed stressing regimes, highlighting the potential importance of matching the applied in vitro stress parameters to the particular nature of in vivo blood damage involved. Forthcoming work is planned for further analysis and reporting on additional aspects of this study.
Introduction
Implantable, durable Left Ventricular Assist Devices (LVADs) sup- port the heart in its role of maintaining circulation through the human body when the heart alone is not capable of maintaining sufficient flow. Recent technological advances have allowed the development of im- plantable LVAD systems, with the pump located inside the body and the power source located outside the body. Use of such devices is presently a treatment of choice for patients with end-stage heart failure or car- diogenic shock refractory to medical therapy, and has been shown to significantly increase survival, functional status and quality of life of patients [1]. Durable VADs are currently utilized as a bridge to hea
transplantation or more frequently as permanent therapy for patients ineligible for heart transplantation [2]. Less commonly, implantable LVADs have been used to bridge patients to myocardial recovery, per- mitting explant of the LVAD. Long-term therapy applications place ever-increasing demands on the reliability and safety of LVAD systems. Some of the challenges are in optimization of pump design (both in configuration aspects and in material hemocompatibility) for minimal blood damage [3]. Center for Devices and Radiological Health (CDRH) requires premarket bench testing for most blood-contacting cardiovascular devices, to determine their potential for causing blood damage. (“Blood damage” refers to a cumulative effect of multiple interrelated perturbations of cellular
Corresponding author at: Functional Fluidics, 440 Burroughs St., Ste. 641, Detroit, MI 48202, USA. E-mail address: michael@functionalfluidics.com (M. Tarasev).
https://doi.org/10.1016/j.ppedcard.2020.101198 Received 9 November 2019; Received in revised form 11 January 2020; Accepted 14 January 2020
Availableonline20January2020 1058-9813/©2020ElsevierB.V.Allrightsreserved.
blood components by various causes, potentially culminating in he- molysis and/or impaired blood cell function, and can involve the coa- gulation cascade, accelerated cleavage of von Willebrand factor, etc.) However, a lack of standardized testing and quantification of “blood damage” causes uncertainties in evaluation of such devices. As noted by the FDA, “it has not been shown that current preclinical hemo- compatibility testing can adequately and reliably predict a device's clinical performance” [4].
Hemolysis is increasingly recognized as a major complication of mechanical circulatory support [5,6] and is strongly associated with patient mortality [7]. Long-term circulatory support involves prolonged contact and collision between blood cells and foreign surfaces, and very high mechanical stresses caused by the pump's impeller (with very high rotation speed) and flow diffuser. Those can cause significant damage to a patients' red blood cells (RBC), particularly due to the strong contribution of turbulent flow stress typical for such devices [8].
Clinical data from patients on circulatory support devices has shown alterations of blood rheology, such as increased blood viscosity and RBC aggregation and decreased RBC deformability [9–12]. These observa- tions further support the prevalence of RBC damage short of immediate hemolysis (also known as “sub-lethal” cell trauma), and hence early RBC removal from circulation. Sub-lethal RBC damage was also cited as a cause of decreased microperfusion and hypoxic RBCs, leading to end organ dysfunction caused by cellular ischemia [13]. It was also im- plicated in shortened cell life span [14], increased trapping in spleen [15], and general deterioration in mechanical properties of the cells [16]. Release of hemoglobin can induce a cascade of effects, starting with decreasing nitric oxide (NO) availability, which can result in im- paired microcirculation and diminished tissue oxygen supply [17,18]. Notably, even low levels of cell-free hemoglobin can drastically in- crease RBC aggregation (especially at low shear conditions [19]), leading to thrombosis. Although the progression from hemolysis to LVAD thrombosis is not yet fully understood, hemolysis is often ac- cepted as an indirect marker for thrombus. Chronic anemia has also been observed in patients supported with mechanical circulatory assist devices, and linked to adverse outcomes in patients receiving prolonged support [20]. While initially anemia is associated with cell hemolysis directly resulting from the mechanical action of the implanted device, chronically it may persist even without detectable atypical induced hemolysis [21], possibly due to a decreased lifespan of circulating RBC due to sub-lethal cell trauma [12]. Overall, device-induced blood trauma remains arguably the biggest barrier to a more widespread use of LVAD technology.
Measurement of pre-existing hemolysis (either direct or indirect through metrics like LDH/LDH1) remains one of the main ways to as- sess blood damage induced by an implanted device. While reflective of terminal red cell damage, such measurements do not reflect possible sub-lethal damage to RBC – i.e. damage short of overt hemolysis which can nevertheless predispose to later hemolysis, early removal from circulation, and/or impaired RBC function (even under normal phy- siological conditions). Such sub-hemolytic RBC damage can be poten- tially evaluated through RBC deformability, typically assessed using ektacytometry, which reflects cell membrane ability to deform without lysing at sub-cell-lethal stress intensities. It can be assayed for example using LORRCA (RR Mechatronics, Netherlands) [22] or other similar systems [23], or via filtering (e.g. [24]), or using certain artificial mi- crofluidic networks [25,26]. Alternatively, RBC mechanical fragility (MF) is a property that reflects induced RBC lysis levels under poten- tially lethal mechanical stress [27,28]. These two related yet different properties are regulated by different skeleton protein associations, and thus can exhibit significantly different responses to membrane damage [29]. Relationships between cell membrane structure, its changes due to internal or external factors, and resultant stability and deformability under stress, are increasingly but still incompletely understood [30].
Methods of mechanical stress application, and particular resultant type(s) of mechanical stress applied, can significantly in
results [31,32], in addition to quantitative parameters like duration and intensity (for a given type of stressing). Cell environment during testing also plays a role [33]. Clinical applications of MF may require or benefit from assessment of different types of RBC membrane perturbation, and thus a test having stressing parameters that enable sufficient control over multiple contributing aspects of the overall stress on a sample is advantageous [32]. Regarding flow-associated stress, both laminar and turbulent flows have notably been implicated in cell fragmentation with different hemolytic thresholds and mechanisms of flow-induced he- molysis [8,34,35]. This contributes to the challenge of determining the optimal type(s) and other parameters of stress application for MF assays so as to best reflect membrane changes and/or defects that may exist in each particular situation (e.g. during LVAD operation).
the present work, we report key preliminary results of an in- vestigation aimed at assessing the use of RBC MF as a potential metric of blood damage caused by of two different models of implantable VADs, and its potential utility in monitoring post-op VAD performance.
Materials and methods
2.1. Patients
Twenty subjects were recruited from the Center for Circulatory Support at the Frankel Cardiovascular Center, Michigan Medicine (University of Michigan). Twelve (60%) subjects were males and eight (40%) were females. Mean age was 54 ± 15 years, with 11 subjects blood type A (55%) and 8 blood type O. Blood samples were collected preoperatively and promptly after the surgery (targeting 1 h, ± 1 h.), and then at 1 day (1 ± 0.2 days), 1 week (6 ± 1 days), and 4 weeks (29 ± 5 days) following LVAD implantation. Preoperative total patient blood volume was estimated using Nadler method [36], and was
5.5 ± 1 L. There was no known history of pre-existing hemolytic or bleeding disorder in any of the subjects. Study aliquots of blood were collected from blood samples for clinical indications. Informed consent was obtained for each patient. The study protocol conforms to the ethical guidelines of the 1975 Declaration of Helsinki as reflected in approval of the study by the University of Michigan IRB (HUM00106930).
2.2. Standard laboratory analysis
Established laboratory tests were performed by the clinical labora- tory of the University of Michigan Center of Circulatory Support, and for each blood collection included hemoglobin, lactase dehydrogenase total (LDH-T) and isoensymes, with special attention to type 1 (LDH1), haptoglobin, free (serum) hemoglobin, and bilirubin.
2.3. RBC Mechanical Fragility (MF) testing
As indicated, there are many ways MF testing could be im- plemented, with varying results; thus, an approach was used which can be controllably varied according to defined parameters. Bead milling with various sizes of cylindrical beads (vs. spherical) had notably been shown to generate different types of mechanical stress in liquid medium, thus allowing testing of RBC MF under substantially different conditions, with potentially substantially different MF results [32]. This is in addition to other (quantitative) variable stressing parameters such as stressing duration/intervals and bead oscillation frequency and amplitude (contributing to stress intensity) [37]. For the results re- ported here, Blaze Medical Devices (Location) used a proprietary (horizontal) electromagnetic bead mill for the sample stressing step, combined with a proprietary spectral analysis method which allows noninvasive determination of hemolysis level in the sample within the cuvette/tube; these testing elements were described previously (at varying degrees of integration/automation) [37]. Three different stressing regimes employed in this study, using these elements albeit
page 2

with variations as indicated, are presented in Table 1.
MF was characterized via MF “profiling”, with hemolysis being determined non-invasively (per noted method) at 1 min intervals, up to 10 min of cumulative stress duration. Area under the curve (AUC) va- lues at the 3 min and 10 min durations were selected to represent each profile – based on the contribution of most hemolytic portion of sample RBC, and total induced hemolysis, respectively. Cumulative stress magnitude, determined by stress application duration as well as bead oscillation frequency and magnetic field strength, were set so as to achieve over 75% induced hemolysis by 10 min in typical samples. Each sample was tested in triplicate; typical standard deviation (SD) of the results was within 5%.
Citrate-stabilized blood samples were diluted with Additive Solution 3 (AS3) to hemoglobin (Hb) concentration of 0.5 g/dl, effectively re- ducing possible cell-cell interactions. Dilution was verified by a Hemoglobin 201 system from HemoCue (Angelholm, Sweden). When desired (and where indicated in the results), AS3 was supplemented with 4 g/dL serum albumin. Physiological concentrations of albumin had been shown previously to significantly reduce RBC propensity to hemolyze [33]. Under some stress application regimes, its presence was suggested to alter bead end and annulus flows, resulting in quantita- tively and qualitatively altered mechanical stress, with consequently altered RBC response [38]. Thus, while such supplementation was im- pactful for stress generated with the parameters of regime 2, it had minimal to no impact on stress when generated with the parameters of regime 1 (Table 1).
Induced hemolysis was calculated based on the difference in ab- sorbance at 576 nm, a wavelength of oxygenated Hb maximum, and at 685 nm, the local minimum for the oxygenated Hb form. It was ex- pressed as a fraction of free hemoglobin (HBF) relative to total he- moglobin concentration (HbT) according to Formula 1, which included the correction for sample hematocrit as detailed by Sowemino-Coker [39].
Total hemoglobin concentration for each diluted RBC sample was determined by subjecting a small (50–100 μL) aliquot to ultrasound for 15 s (0.1 s pulses, with 0.2 intervals between pulses, on ice) delivered by a Branson Digital Sonifier 450 (Danbury, CT), at 15% intensity (from the manufacturer specified 400 W). In control experiments, such treatment was shown to fully lyse RBC without inducing hemoglobin oxidation. For determining auto-hemolysis (i.e., hemolysis prior to any application of mechanical stress), small (30–50 μL) aliquots of un- diluted samples were centrifuged for 5 min at 5000 rpm, supernatants were collected, and hemoglobin content was measured spectro- photometrically.
2.4. Materials
Bovine serum albumin (BSA) was purchased from RPI Corp (Mt. Prospect, IL); all other reagents were purchased from Sigma-Aldrich (St. Louis, MO) and Fluka (Honeywell, Morristown, NJ).
2.5. Statistical methods
Mixed effects models were used to evaluate the data. When appro- priate, time of collection (Pre-, Post-, 24-h, 7-day, 1-month sample collection points) was used as predictors; multiple observations over time per subject were accounted for through covariance matrix of the residuals, so that p-values for fixed effect were adjusted for “within- subjects” (same-subject) correlation, except when appropriate, single time points were used with linear regression models. Values are pre- sented as mean ± standard deviation. Distributions are shown using Box-and-Whisker plot, with the box showing upper and lower quartiles, the median, and the mean (X), and lower and upper whiskers showing at 1.5 x IQR (interquartile range), with data points (including outliers) shown. A p-value ≪ 0.05 was considered significant.
3. Results
3.1. Surgical procedures
Two LVAD systems were used in the study, with 13 of the subjects implanted with the HVAD (Medtronic, Inc., Minneapolis, MN), and 7 with the HeartMate 3® LVAD (HM3; Abbott Labs, Chicago, IL). All implants for both devices were performed through a median ster- notomy incision with cardiopulmonary bypass. The pericardium was mobilized, and a pericardial cradle was created in the usual fashion. The driveline (percutaneous lead) was tunneled from the pericardial space through the right upper quadrant of the abdominal wall and exited 2 to 3 cm below the costal margin at the mid clavicular line prior to administration of heparin for cardiopulmonary bypass. The patient was placed in Trendelenberg position before cardiopulmonary bypass was initiated. The apical ring was then sewn to the apex of the left ventricle using horizontal pledgetted mattress sutures with 2–0 Ethibond in an interrupted fashion. After securing the apical ring to the
apex of the left ventricle, an apical core of the left ventricular musc
was removed using a coring knife. The inlet cannula of the LVAD wthen positioned within the apical connector and secured in the usual fashion. The ventricle was partially filled to evacuate air through the outflow conduit of the LVAD. Next, a side-biting vascular clamp was placed on the ascending aorta and an aortotomy sized to the outflow graft was made in the ascending aorta. The outflow graft was sized and then sewn to the ascending aorta in an end-to-side fashion using 5–0 Prolene suture in a running fashion.
olene suture in a running fashion. Average cardiopulmonary bypass (CPB) times were significantly (P < 0.05) longer for HM3, than for HVAD and constituted 126 ± 67 (67–220) min for HM3 and 74 ± 14 (51–96) min for HVAD. Four HVAD patients and 2 HM3 patients (about 30% for both devices) had tricuspid valve repair (TVR). The rate of utilization of implant con- comitant procedures was similar between the two devices (Table 2). This difference arises from CPB times for 3 HM3 having procedures lasting for 162, 202 and 220 min, as compared to the other 4 HM3 implantation that had CBPs between 67 and 85 min, comparable to those in HVAD. Elevated CPB times in HM3 correlated with an increase in mechanical fragility (HLA-10 and HLA-3 metrics, P = 0.06, R2 = 0.7, Fig. 1a) and bilirubin (P ≪ 0.01, R2 = 0.95, Fig. 1b), but not with changes in LDH1, haptoglobin or serum hemoglobin. For RBC MF, the correlation was predicated on the single largest CPB value for one of the
page 3
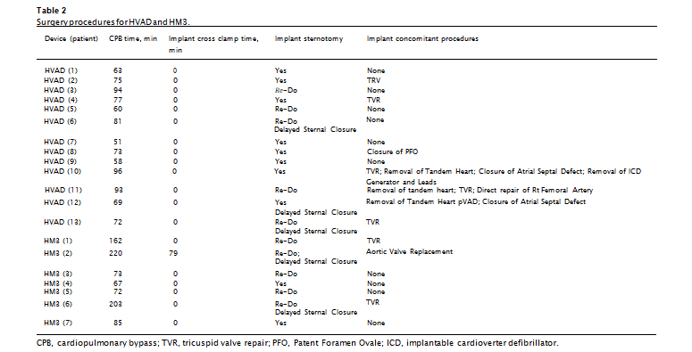
HM3 patients (220 min), and if such was excluded from the analysis, correlation of CPB times with MF is no longer significant. This indicates the need for a larger sample size for comprehensive assessment of the impact of CPB time.
3.2. Clinical outcomes
Two patients developed complications within their observation period (30 days post-surgery), both exhibiting Cardiac Arrhythmia – and in one case combined with post-surgery bleeding, infection and pleural effusion (10 days after surgery). The small sample size of sub- jects having complications did not allow meaningful analysis of possible correlations with the various hematological parameters measured in this study (including RBC MF).
3.3. Transfusion
During surgery, in addition to volume expanders, subjects received transfusions with whole blood (WB), packed RBC (pRBC), fresh frozen plasma (FFP) and platelets, both intraoperatively and postoperatively (Table 3). Additionally, 3 subjects received small volumes (240, 440, and 440 ml) of cell saver blood. Five of the subjects (3 with HVAD, and 2 with HM3) accounted for approximately 80% of all pRBC and FFP transfusions, but only approximately 25% of WB transfusions. With the exception of crystalloids, the differences between the volume of blood component therapy administered with either the HVAD or HM3 im- plantation procedures were not statistically significant (Table 3). Sample size of the cell saver blood transfusions precluded meaningful statistical analysis for that variable. Between the two devices, differ- ences in blood loss during surgery were not significant. Whole Blood transfusions for HVAD and pRBC and plasma transfusions for HM3 were positively correlated with cardiopulmonary bypass (CPB) time.
Due likely to high inter-subject variability, surgery and associated transfusions did not effectuate statistically significant changes in average (over all patients) levels of serum hemoglobin (serum Hb), bilirubin, lactate dehydrogenase −1 (LDH1), and haptoglobin. However, pairwise comparison showed that an observ
2.5. Statistical methods
levels of serum Hb, bilirubin, LDH1, and decrease in haptoglobin, were indeed statistically significant with p-value (P) < 0.05. Multiple re- gression analysis showed that transfusion and surgery-related changes in LDH1 were significantly correlated with the amount of whole blood (WB) transfusions, with R2 ≈ 0.36. When in combination with the number of units of pRBC and FFP transfused, the model's explanatory significance of LDH1 variability increased up to 58% with P ≪ 0.05 (combination of WB, pRBC, and FFP used as predictor variables). WB and pRBC were positively correlated with LDH1, while for FFP the model showed a negative correlation. No correlation was observed between transfused amounts of WB, pRBC, and FFP and patient con- dition metrics bilirubin, serum hemoglobin and haptoglobin./p>
3.4. Device type
There was no statistically significant difference between basic pre- surgery laboratory values for patients implanted with the HVAD or HM3 (Table 4). LDH1, serum hemoglobin, and bilirubin all increased after surgery, with such increases reaching statistical significance at 1 h after surgery for serum hemoglobin and bilirubin. LDH1 increase was statistically significant at 24 h after surgery (P < 0.05), concurrently with a further increase (P < 0.1) of bilirubin, and a decrease in serum hemoglobin (Fig. 2). Haptoglobin was decreased (P < 0.1) at 1 h after surgery, and at 7 days increased from its 1 h and 24 h after surgery le- vels (P < 0.05). LDH1 remained elevated at 7 days after surgery and decreased (P < 0.05) along with bilirubin (P < 0.1) 30 days after surgery, with changes in serum hemoglobin and haptoglobin not reaching statistical significance.Mixed effects models showed device type as a statistically sig- nificant predictor of post-surgery LDH1 levels, with such being about 13% higher overall for HM3. No correlation was observed for other potential hemolysis metrics (serum hemoglobin, bilirubin and hap- toglobin), total LDH, or other LDH isoenzymes. With device type used as a predictor, linear regression analysis demonstrated a R2 value of 0.3 (model predictive capability of 30%) at P = 0.02 for LDH1 changes between 1 h and 24 h after surgery, and R2 = 0.2 (P = 0.06) for changes between 24 h and 7 days after surgery (Fig. 3).
page 4
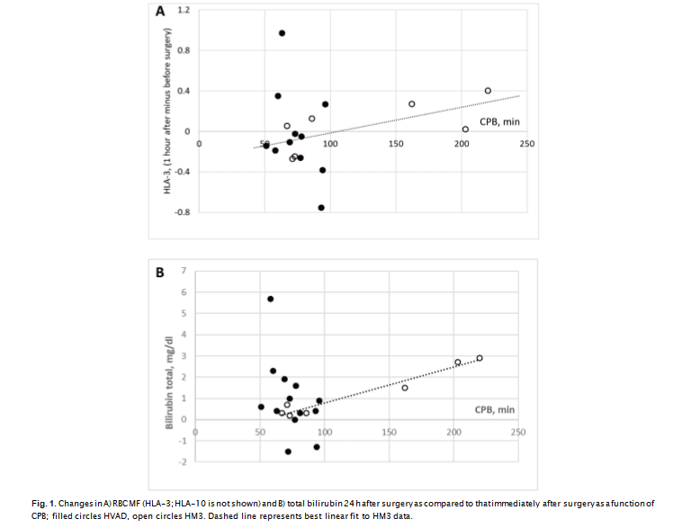
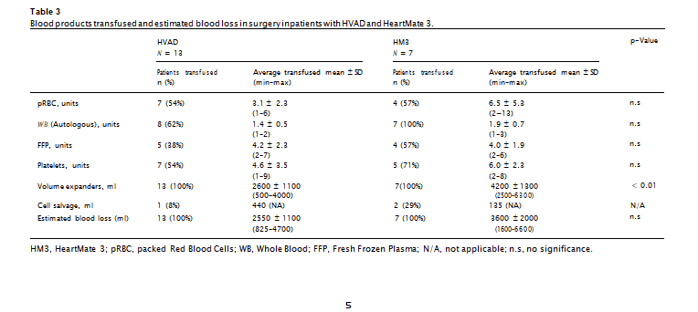
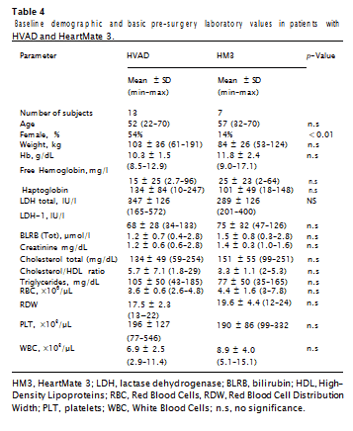
not a statistically significant predictor of LDH1 at 30 days after surgery. For the full 20-patient population, RBC MF results with non-al- bumin-supplemented sample media showed a slight decline (significant at P < 0.1) at 1 day after surgery, with the follow-up changes not statistically significant. For the same population, average MF whe tested in the presence of albumin increased after surgery up to 1 week after surgery, and then declined to about pre-surgery level at 4 weeks after surgery. For both the most labile (fragile) fraction and the overall cumulative RBC MF, the increase was statistically significant at P < 0.1, with the follow-up decrease significant at p-value < 0.1 for HLA-3 and P < 0.05 for HLA-10
nificance for device type as a predictor variable (at P < 0.05 for 2 and 7 days after surgery, and P < 0.1 for “immediately” (~1 h) afte surgery). HM3 was associated with higher MF values, with about
15–20% difference in MF overall. Device type was not a significant predictor of RBC MF at 30 days after surgery. It also was not a sinificant predictor of MF at any of sample collection time points when measured using AS3 not supplemented with albumin (HL-3 and HL-10 indices, and HS-3 and HS-10 indices, Fig. 4); the excepti shown to be a statistically significant (P < 0.05) predictor of me- chanical fragility
Linear regression analysis shows that device type predicted about 20% and 30% of the variability for HLA-3, and 26% and 51% of the variability in HLA-10, for 7 and 30 days (respectively). For MF mea sured at ~1 h after surgery, such predictive capability was 17% and 19% for HLA-3 and HLA-10, respectively (at 0.05 < P < 0.1).
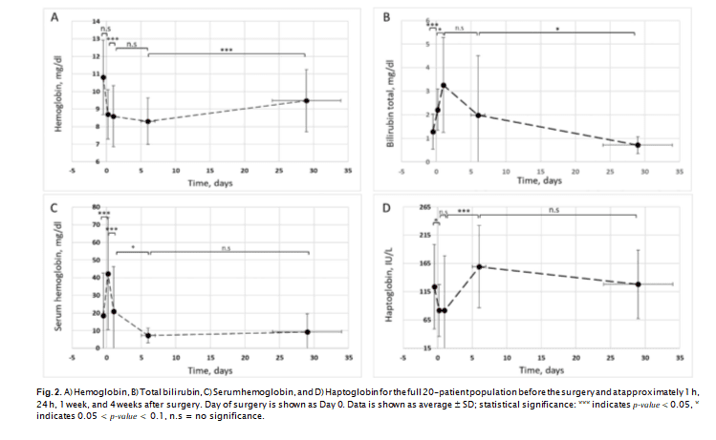
page 6
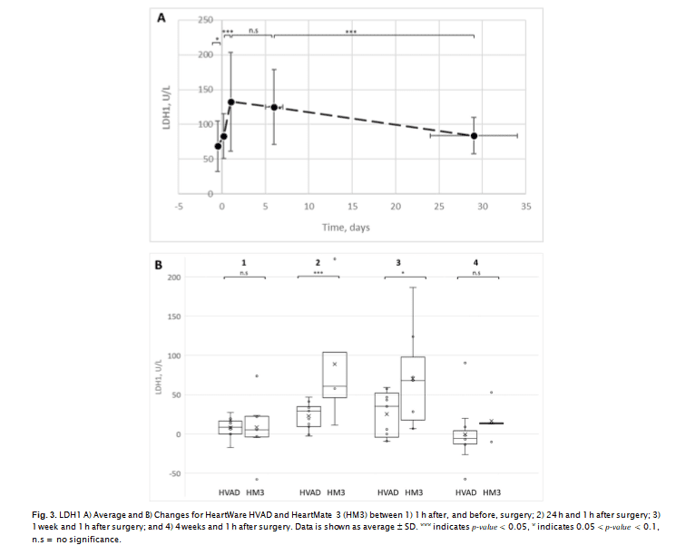
4. Discussion
As indicated above, long-term therapy applications place ever-in- creasing demands on reliability and safety of LVAD systems. Thus, de- velopment of alternative or supplemental metrics to assess blood da- mage remains a priority both for device development and for monitoring and comparing devices' performance post-implantation.
Performance of the continuous flow pumps with centrifugal design (i.e. HVAD and HM3 LVAD) are commonly compared to the continuous flow pumps with axial design (i.e. HeartMate II, HMII), which until recently was the most frequently implanted durable LVAD in the United States. The HMII is an axial flow pump with a central ruby bearing supporting the rotor. It operates at higher, pump speed (typically at 9000 rpm, with 6000 rpm minimum) compared to centrifugal systems [40]. A key difference between the designs is that axial-flow pumps like HM2 (Fig. 5) have the impeller outflow directed parallel to the axis of rotation, while in the centrifugal pumps the outflow is directed per- pendicular to the axis of rotation.
The HVAD and the HM3 devices both utilize centrifugal flow de- signs using non-contact levitation of the internal impellor, reflecting design considerations to aid hemocompatibility (minimization of he- molysis and thrombosis). Both systems also allow for sequential
changes in pump speed to induce flow pulsation (with the aim of re- ducing possible thrombosis), which a number of studies linked to better long-term clinical outcomes [41]. The HVAD system (Fig. 6) utilizes a spinning, wide-blade impeller suspended with a hybrid levitation system combining a hydrodynamic bearing (a layer of blood is needed to lift the rotor inside the pump) and passive magnetic levitation with a rare-earth magnet. Its short inlet cannula and bearing-less impeller with primary, secondary and tertiary blood flow paths is thought to reduce the blood transit time through the device to enhance blood flow and reduce device-induced blood trauma [42]. Typical operating speed is between 2400 and 3200 rpm [43], with the average speed in this study (2700 ± 200 rpm) falling within that range.
The HM3 system (Fig. 7) is designed with a fully magnetically le- vitated rotor, wide flow gaps, and textured surfaces [44]. Use of full magnetic levitation means that no blood bearing is needed to levitate the rotor, eliminating that possible source of blood damage. Typical operating speed is between 4000 and 6000 rpm (5300 ± 200 rpm in this study). Of the two systems, the HM3 is the newer design and has enlarged gaps along the side, top and bottom of the impeller and the pump housing and impeller.
Overt hemolysis and other blood damage indicators had notab
page 7
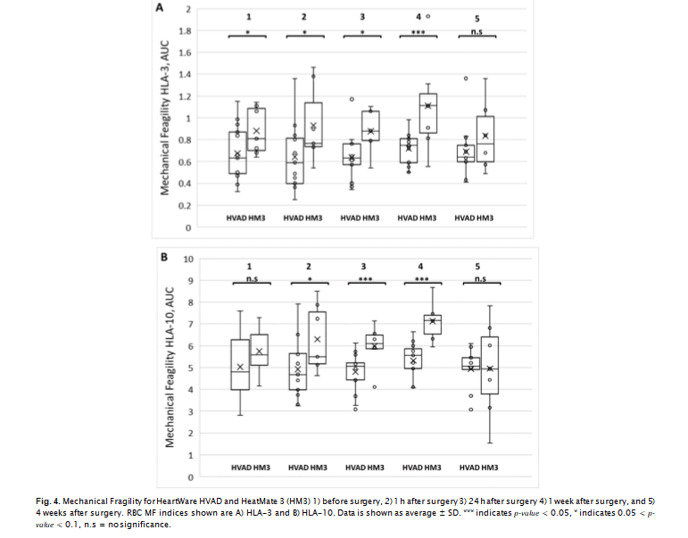
been areas of LVAD investigation. A published 20-patient study, which compared HMII and the HVAD, found that hemolysis as reflected via LDH appeared more pronounced in patients with the HMII compared to the HVAD [45,46]. However, while quality of life and functional ca- pacity increased about equally for both devices, and HVAD experienced less malfunctions and failures, the rates of stroke (ischemic or hemor- rhagic) were found to be significantly higher in the newer centrifugal HVAD than in the older axial HM2 [47]. However, recently published analysis of available data seems to indicate that with strict post- operative blood pressure control, the HVAD has similar outcomes as the HM2 with respect to stroke, and improved performance with respect to pump thrombosis [48,49].
In the MOMENTUM 3 trial, HM3 was compared with HMII for “hemocompatibility-related outcomes” and received a better (lower) overall “hemocompatibility score,” including a complete absence of pump thrombosis (in contrast to HM2) as well as lower stroke rates over the 2-year period [50].
Head-to-head comparison between HMII, HM3, and HVAD has shown no significant difference in patient survival and comparable results for length of hospital stay, inflammatory parameters, and rates of most postoperative complications; however, HM3
with substantially higher (47.1%, P = 0.056) need of postoperative onset of dialysis [51]. Those results should be considered with the understanding that the data included surgeries performed over a 10- year period, with potential changes in pre- and post-operative proce- dures. Possible selection bias, based on patient condition, especially in the early years, also cannot be excluded.
In the current study, HM3 showed elevated, compared to HVAD, levels of hemolysis as assessed through LDH1 for up to 1 week after surgery. HM3, as compared to HVAD, was also associated with de- creased RBC stability, as evidenced by elevated RBC propensity to lyse under applied stress (elevated RBC MF). Notably, for both devices, at 4 weeks after surgery all metrics reverted to about their pre-surgery levels, with no statistically significant differences between the two time points (even for serum hemoglobin, with its decline at 1 and 4 weeks still not reaching statistically significance, due to high variability of its pre-surgery levels).
It should be noted, that cardiopulmonary bypass by itself can be the cause of additional blood damage. About half of HM3 patients had CPB times much longer than the maximum CPB time recorded for HVAD. It is possible, that such elevated CPBs may be contributing factors for the observed early differences in MF and hemolysis. Interest
Page 8
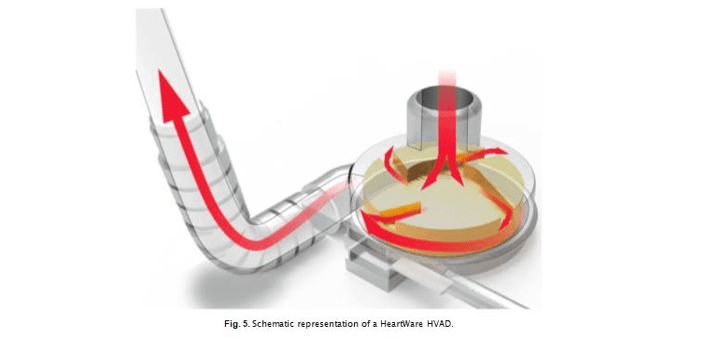
CBP times correlated with elevated MF at 24 h after surgery but not with LDH1. Considering overall changes in LDH1 concentration, which increased at 24 h and remained essentially unchanged from that after 7 days, it can be concluded that while CPB times may be an important contributor to the observed differences in RBC damage, they are not the only contributing factor for hemolysis. Additionally, even though the differences between HVAD and HM3 in the amounts of transfused products were not statistically significant, reported correlations of some of those with CPT times suggest the need to further evaluate transfusion impact on early changes in RBC MF and hemolysis.
Depending on the type of external mechanical stress, the RBC membrane can be subject to different types of deformation, including both those associated with bending and/or torsional deformations and membrane compression-stretching. While the membrane can tolerate great deformations if its surface area remains unchanged, it is very sensitive to changes in membrane surface area, with even a sma
compression-stretching resulting in cell fragmentation. All these could be expected to affect RBC membrane stability in different ways. Hemolysis can occur both in-bulk (including through cell-cell interac- tions and by direct shear) as well as at solid surfaces and air interfaces (e.g. [34,52,53]). In laminar flows, the stress across the membrane would be determined by the flow around the cell and relate to velocity gradient and fluid viscosity. In turbulent flow, it was suggested that cell damage can arise through eddies comparable to cell size [54,55]. However, even with attempts to account for actual turbulence structure and cell microenvironment, prediction of flow-induced cell damage is complicated and may still be inadequate [56]. Such reports highlight the difficulties in predicting and analyzing flow-induced hemolysis can be further complicated by cell interaction with blood proteins and biological or artificial surfaces. It was suggested that for RBC suspen- sions in buffer solutions, turbulent flows may be expected in the bead annulus, although for certain bead/tube configurations this turbulence
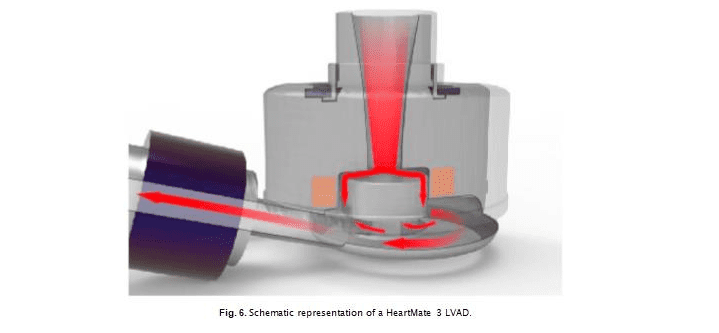
Page 9
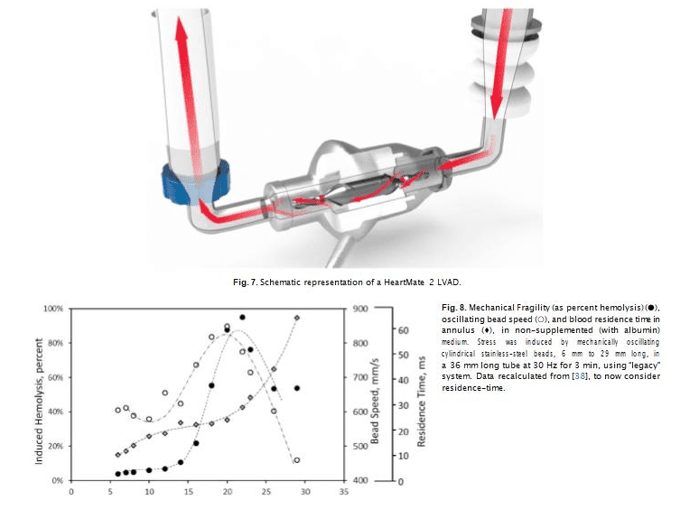
may be suppressed [32]. Also albumin supplementation resultants in an increase in viscosity and associated changes in flow velocity, which under certain conditions may make annulus turbulence less favorable and facilitate a transition of at least part of the flow to a laminar fashion. Notably, with a cylindrical bead, flow may also vary across the width of the annulus due to flow speed transition away from a sta- tionary outer tube wall.
For VADs, shear-induced hemolysis is driven by both stress magni- tude/intensity and by blood residence/exposure time to stress. Depending on the system type, one of these contributing factors may become dominant, with the biggest difference being between axial (higher speed/shorter residence time) and centrifugal (lower speed/ longer residence time) pumps [57].
With bead milling, changes in flow speed and time under stress could be modeled by alteration of bead dimensions. For a given bead (and thus annulus) length, flow speed would be directly correlated to residence time, while for beads of a given diameter, residence time would be affected directly by length of annulus and indirectly the an- nulus length's impact on flow speed. We previously reported (albeit using a different, “legacy” bead-milling approach) that magnitude of induced hemolysis indeed depends significantly on relative (to the tube/container) dimensions of the oscillating cylindrical bead; specifi- cally, for a given bead diameter and thus a given size of annulus gap, hemolysis was well correlated with the speed of the oscillating bead [38]. Bead (and thus blood flow) speed and induced hemolysis ha
similar dependence on annulus length – while residence time, a func- tion of both annulus length and speed of blood flow, appear to have exhibited a dependence on annulus length dissimilar from that of in- duced hemolysis (Fig. 8). That suggests that, at least for the system used in that study, flow speed and not residence time was the dominant factor in RBC lysis.
Albumin, and other blood plasma proteins to a lesser degree, can significantly influence magnitudes of mechanically induced hemolysis; specifically, we've previously demonstrated that with bead milling using standard ball/spherical beads, albumin can provide marked “protection” against mechanically induced hemolysis [33]. There, the magnitude of such protection was shown to depend on flow char- acteristics, but for samples passing through a substantial annulus (as is the case when using cylindrical beads [32]), it is also a function of annular length and width. For a given annulus width, we've previously found that higher speeds were correlated with a larger albumin-related decrease in induced hemolysis, and lower induced lysis overall in the presence of albumin [38]. For cylindrical beads of different diameters, the minimum induced hemolysis was observed at a 0.8 ratio of bead to container (tube) diameters (RBD/TD), corresponding for that geometry with 0.88 mm. Higher hemolysis was observed at both larger (up to
2.3 mm, RBD/TD = 0.91) and smaller (down to 0.4 mm, RBD/TD = 0.57) gap widths [38]. These trends were markedly more pronounced in al- bumin supplemented medium, with relative magnitudes varying be- tween blood from different donors. This donor-to-do
Page 10
could have been due to variability in plasma lipids, which had been shown previously to significantly reduce RBC stability under mechan- ical stress [58,59].
Of the three mechanical stress application regimes reported here that were used in the present study, only one showed a difference in RBC MF between the HVAD and HM3. This highlights both the need and the potential for controllable differentiation of hemolysis-inducing stress, and optimal selection/manipulation of the various bead milling parameters. One possible approach is through modeling bead milling flow based on that of particular VADs. It remains to be demonstrated, computationally and/or experimentally, how changes in the oscillating bead's geometry or design could be targeted to generate flows suffi- ciently correlating to those in VADs, and clinically, whether such ex- vivo testing would indeed correlate with VAD outcomes. Notably, characteristics of such flows would be expected to differ across different VAD (or other) device types, thus potentially necessitating device-spe- cific selection of bead milling parameters.
Forthcoming work is planned for further analysis and reporting on additional aspects of the present study
4.1. Considerations for pediatric application
Implementation of LVAD support for pediatric patients has histori- cally lagged behind that for adults. Congenital Heart Disease (CHD) physiology, the small size of device required, and complexities of pe- diatric anticoagulation had been cited as main reasons for the disparity of adoption rates [60]. Berlin Heart Excor (Berlin Heart GmbH, Berlin, Germany), an external pulsatile, pneumatically-driven VAD, was the first system approved by the FDA for children in 2005. With the ex- ception of the Jarvik 15 mm, none of the currently available im- plantable LVADs had been specifically designed for pediatric applica- tion. (Jarvik 15 mm is a fully implantable, continuous flow LVAD, redesigned from Infant Jarvik 2000, which in testing demonstrated unacceptably high levels of hemolysis – a complication that required 2 years to remedy [61].) Jarvik 15 mm is currently undergoing clinical trials expected to conclude in 2022.
Present use of VADs in the pediatric population shows a clear age- dependence, which complicates the comparison of performance be- tween devices, due to large differences in patient size and ongoing changes in physiology. Moreover, while extracorporeal circulatory support devices like ECMO and extracorporeal pumps such as the CentriMag (> 10 kg)/Pedimag (< 10 kg) are suitable for any size pa- tients as a bridge to transplant, Berlin Excor remains the only option for the smallest patients, with adult-size HVAD systems being limited mostly to children of school age, with the concern that for smaller ones the required flow rate may become too low – thus elevating the risks of pump thrombosis. Still, similar to with the adult population, use of circulatory support devices for destination therapy in children is ap- pealing, particularly in light of the possible decline in the need for such support as a child age.Based on the Second Annual Pediatric Interagency Registry for Mechanical Circulatory Support (Pedimacs) report, out of 364 proce- dures that year, Implantable Continuous (IC) VADs, namely the HVAD and HMII as listed, were the most often used (close to 50% of cases), followed by Paracorporeal Pulsatile (PP), like Berlin Heart (~ 30%), and Paracorporeal Continuous (PC), like Centrimag/Perimag or Maquet Rotaflow (16%). It was reported that device selection differed sig- nificantly by the age of the patient, with the median age for both PP and PC devices at 1.7 years as compared to 14.9 years for IC devices [62]. This ratio between device types does not change significantly for the next year, with a small (about 10%) increase in the total annual number of the procedures. It was reported that positive outcomes for IC VADs exceed 90% at 6 months [63]. It can be anticipated that in the near future, HeartMate 3 may also find utility in pediatric applications, especially for older (adolescent) children. Hopefully, introduction of new devices, designed and validated for pediatric use,
further improvements in success rates for children in need of circulatory support.
Acknowledgements
The study reported in this article was funded by a Phase 1 SBIR grant from the FDA (Grant #1R43FD005472-01) for a joint project between Blaze Medical Devices and the University of Michigan. Illustrations of the devices were custom made for Functional Fluidics. The authors would like to acknowledge Randall Bath and Marina Muchnik for lab assistance with the RBC MF testing and associated tasks, as well as Lydia McGowan and Alondra Dorsey for their assis- tance with IRB submission and study coordination.
Disclosure
Authors Tarasev, Chakraborty, and Alfano were employees of, and hold equity in, Blaze Medical Devices – a company that developed the RBC MF testing technology used in this work. Author Alfano con- tributed to the work solely in his capacity with Blaze. Author Tarasev is presently employed with Functional Fluidics – a company that develops methods for assessment of cell function, and which now holds an ex- clusive license in the technology developed by Blaze
References
- Frazier OH, Rose EA, Oz MC, et al. Multicenter clinical evaluation of the HeartMate vented electric left ventricular assist system in patients awaiting heart transplan- tation. J Thorac Cardiovasc Surg 2001;122:1186–95.
- Kirklin JK, Naftel DC, Kormos RL, et al. Second INTERMACS annual report: more than 1,000 primary left ventricular assist device implants. J Heart Lung Transplant 2010;29. /li>
- Schmid Daners M, Kaufmann F, Amacher R, et al. Left ventricular assist devices: challenges toward sustaining long-term patient care. Ann Biomed Eng 2017;45:1836–51./li>
- FDA. 2012.
- Ravichandran AK, Parker J, Novak E, et al. Hemolysis in left ventricular assist de- vice: a retrospective analysis of outcomes. J Heart Lung Transplant 2014;33:44–50. [
- Cowger JA, Romano MA, Shah P, et al. Hemolysis: a harbinger of adverse outcome after left ventricular assist device implant. J Heart Lung Transplant 2014;33:35–43.
- Ravichandran A, Parker J, Joseph S, et al. Hemolysis Is Strongly Associated with Mortality in LVAD Patients. J Heart Lung Transplant 2013;32:S56.
- Kameneva MV, Burgreen GW, Kono K, Repko B, Antaki JF, Umezu M. Effects of turbulent stresses upon mechanical hemolysis: experimental and computational analysis. ASAIO J 2004;50:418–23.
- Kormos RL, Borovetz HS, Griffith BP, Hung TC. Rheologic abnormalities in patients with the Jarvik-7 total artificial heart. ASAIO Trans 1987;33:413–7.
- Frattini PL, Wachter C, Hung TC, Kormos RL, Griffith BP, Borovetz HS. Erythrocyte deformability in patients on left ventricular assist systems. ASAIO Trans 1989;35:733–5.
- Hung TC, Borovetz HS, Kormos RL, et al. Artificial heart. Hemorrheology and transient ischemic attacks. ASAIO Trans 1990;36:M132–5.
- Pierce CN, Larson DF. Inflammatory cytokine inhibition of erythropoiesis in pa- tients implanted with a mechanical circulatory assist device. Perfusion. 2005;20:83–90.
- Vercaemst L. Hemolysis in cardiac surgery patients undergoing cardiopulmonary bypass: a review in search of a treatment algorithm. J Extra Corpor Technol 2008;40:257–67.
- Brinsfield DE, Hopf MA, Geering RB, Galletti PM. Hematological changes in long- term perfusion. J Appl Physiol 1962;17:531–4.
- Sandza JG, Jr., Clark, RE, Weldon, CS, and Sutera, SP. Subhemolytic trauma of erythrocytes: recognition and sequestration by the spleen as a function of shear. Trans Am Soc Artif Intern Organs. 1974; 20 B: 457–462.
- Baskurt OK, Uyuklu M, Meiselman HJ. Protection of erythrocytes from sub-hemo- lytic mechanical damage by nitric oxide mediated inhibition of potassium leakage. Biorheology 2004;41:79–89.
- Minneci PC, Deans KJ, Zhi H, et al. Hemolysis-associated endothelial dysfunction mediated by accelerated NO inactivation by decompartmentalized oxyhemoglobin. J Clin Invest 2005;115:3409–17.
- Rother RP, Bell L, Hillmen P, Gladwin MT. The clinical sequelae of intravascular hemolysis and extracellular plasma hemoglobin: a novel mechanism of human disease. JAMA. 2005;293:1653–62.
- Seiyama A, Suzuki Y, Maeda N. Increased viscosity of erythrocyte suspension upon hemolysis. Colloid Polym Sci 1993;271:63–9.
- Vrtovec B, Radovancevic R, Delgado RM, et al. Significance of anaemia in patients with advanced heart failure receiving long-term mechanical circulatory support. Eur J Heart Fail 2009;11:1000–4.
- Pierce CN, Larson DF, Arabia FA, Copeland JG. Inflammatory mediated chronic anemia in patients supported with a mechanical circulatory assist device. J Extra Corpor Technol 2004;36:10–5.
Page 11
- Zaninoni A, Fermo E, Vercellati C, et al. Use of laser assisted optical rotational cell analyzer (LoRRca MaxSis) in the diagnosis of RBC membrane disorders, enzyme defects, and congenital dyserythropoietic anemias: a monocentric study on 202 patients. Front Physiol 2018;9:451.
- Baskurt OK, Hardeman MR, Uyuklu M, et al. Comparison of three commercially available ektacytometers with different shearing geometries. Biorheology. 2009;46:251–64.
- Odashiro K, Saito K, Arita T, Maruyama T, Fujino T, Akashi K. Impaired deform- ability of circulating erythrocytes obtained from nondiabetic hypertensive patients: investigation by a nickel mesh filtration technique. J Clin Hypertens 2015;21:17.
- Stauber H, Waisman D, Korin N, Sznitman J. Red blood cell dynamics in biomimetic microfluidic networks of pulmonary alveolar capillaries. Biomicrofluidics 2017;11:014103.
- Cluitmans JCA, Chokkalingam V, Janssen AM, Brock R, Huck WTS, Bosman GJCGM. Alterations in red blood cell deformability during storage: a microfluidic approach. Biomed Res Int 2014;2014:9.
- Baskurt OK, Meiselman HJ. Red blood cell mechanical stability test. Clin Hemorheol Microcirc 2013;55:55–62.
- Kameneva MV, Antaki JF. Mechanical trauma to blood. In: Baskurt OK, editor. Handbook of Hemorheology and Hemodynamics. Netherlands, Amsterdam: IOS Press; 2007. p. 206–27.
- Chasis JA, Mohandas N. Erythrocyte membrane deformability and stability: two distinct membrane properties that are independently regulated by skeletal protein associations. J Cell Biol 1986;103:343–50.
- Viallat A, Abkarian M. Red blood cell: from its mechanics to its motion in shear flow. Int J Lab Hematol 2014;36:237–43.
- Gu L, Smith WA, Chatzimavroudis GP. Mechanical fragility calibration of red blood cells. ASAIO J 2005;51:194–201.
- Alfano KM, Chakraborty S, Tarasev M. Differences in bead-milling-induced hemo- lysis of red blood cells due to shape and size of oscillating bead. Biomed Mater Eng 2016;27:405–12.
- Tarasev M, Chakraborty S, Light L, Davenport R. Impact of environment on red blood cell ability to withstand mechanical stress. Clin Hemorheol Microcirc 2016.
- Sutera SP. Flow-induced trauma to blood cells. Circ Res 1977;41:2–8.
- Yen JH, Chen SF, Chern MK, Lu PC. The effect of turbulent viscous shear stress on red blood cell hemolysis. J Artif Organs 2014;17:178–85.
- Nadler SB, Hidalgo JH, Bloch T. Prediction of blood volume in normal human adults. Surgery. 1962;51:224–32.
- Alfano KM, Tarasev M, Meines S, Parunak G. An approach to measuring RBC haemolysis and profiling RBC mechanical fragility. J Med Eng Technol 2016;40:162–71.
- Tarasev M, Muchnik M, Chakraborty A. Impact of the oscillating bead size and shape on induced mechanical stress on red blood cells and associated hemolysis in bead milling. Int J Blood Res Disord 2019;6:41.
- Sowemimo-Coker SO. Red blood cell hemolysis during processing. Transfus Med Rev 2002;16:46–60.
- Noor MR, Bowles C, Banner NR. Relationship between pump speed and exercise capacity during HeartMate II left ventricular assist device support: influence of residual left ventricular function. Eur J Heart Fail 2012;14:613–20.
- Cheng A, Williamitis CA, Slaughter MS. Comparison of continuous-flow and pul- satile-flow left ventricular assist devices: is there an advantage to pulsatility? Ann Cardio Surg 2014;3:573–81.
- Tuzun E, Roberts K, Cohn WE, et al. In vivo evaluation of the HeartWare centrifuga ventricular assist device. Tex Heart Inst J 2007;34:406–11.
- Haglund NA, Davis ME, Tricarico NM, et al. Perioperative blood product use: a comparison between HeartWare and HeartMate II devices. Ann Thorac Surg 2014;98:842–9.
- Bourque K, Cotter C, Dague C, et al. Design rationale and preclinical evaluation of the HeartMate 3 left ventricular assist system for hemocompatibility. ASAIO J 2016;62:375–83.
- Birschmann I, Dittrich M, Eller T, et al. Ambient hemolysis and activation of coa- gulation is different between HeartMate II and HeartWare left ventricular assist devices. J Heart Lung Transplant 2014;33:80–7.
- Shah P, Mehta VM, Cowger JA, Aaronson KD, Pagani FD. Diagnosis of hemolysis and device thrombosis with lactate dehydrogenase during left ventricular assist device support. J Heart Lung Transplant 2014;33:102–4.
- Rogers JG, Pagani FD, Tatooles AJ, et al. Intrapericardial left ventricular assist device for advanced heart failure. N Engl J Med 2017;376:451–60.
- Florisson DS, Conte SM, De Bono JA, Newcomb AE. Do patients with the centrifugal flow HeartMate 3 or HeartWare left ventricular assist device have better outcomes compared to those with axial flow HeartMate II? Interact Cardiovasc Thorac Surg 2019.
- Milano CA, Rogers JG, Tatooles AJ, et al. HVAD: the ENDURANCE supplemental trial. JACC Heart Fail 2018;6:792–802. [50] Uriel N, Colombo PC, Cleveland JC, et al. Hemocompatibility-related outcomes in the MOMENTUM 3 trial at 6 months. Circulation. 2017;135:2003–12.
- Zhigalov K, Mashhour A, Szczechowicz M, et al. Clinical outcome and comparison of three different left ventricular assist devices in a high-risk cohort. Artif Organs 2018;42:1035–42.
- Leverett LB, Hellums JD, Alfrey CP, Lynch EC. Red blood cell damage by shear stress. Biophys J 1972;12:257–73.
- Beissinger RL, Laugel J-F. Low-stress hemolysis in laminar blood flow: bulk and surface effects in capillaries. AICHE J 1987;33:99–108.
- Liu JS, Lu PC, Chu SH. Turbulence characteristics downstream of bileaflet aortic valve prostheses. J Biomech Eng 2000;122:118–24.
- Lu PC, Lai HC, Liu JS. A reevaluation and discussion on the threshold limit for hemolysis in a turbulent shear flow. J Biomech 2001;34:1361–4.
- Dooley PN, Quinlan NJ. Effect of eddy length scale on mechanical loading of blood cells in turbulent flow. Ann Biomed Eng 2009;37:2449–58.
- Chen Z, Jena SK, Giridharan GA, et al. Flow features and device-induced blood trauma in CF-VADs under a pulsatile blood flow condition: a CFD comparative study. Int J Numer Methods Biomed Eng 2018;34:e2924.
- Tarasev M, Alfano K, Chakraborty S, Light L, Doeden K, Gorlin JB. Similar donors- similar blood? Transfusion (Paris) 2014;54:933–41.
- Tarasev M, Chakraborty S, Light L, Davenport R. Impact of environment on Red Blood Cell ability to withstand mechanical stress. Clin Hemorheol Microcirc 2016;64:21–33.
- Pinney SP, Chen JM. Pediatric ventricular assist devices. A quarter-century of support. Yet So Far to Go 2018;72:416–8.
- Baldwin JT, Adachi I, Teal J, et al. Closing in on the PumpKIN trial of the Jarvik 2015 ventricular assist device. Semin Thorac Cardiovasc Surg Pediatr Card Surg Annu 2017;20:9–15.
- Blume ED, VanderPluym C, Lorts A, et al. Second annual Pediatric Interagency Registry for Mechanical Circulatory Support (Pedimacs) report: pre-implant char- acteristics and outcomes. J Heart Lung Transplant 2018;37:38–45.
- Morales DLS, Rossano JW, VanderPluym C, et al. Third annual Pediatric Interagency Registry for Mechanical Circulatory Support (Pedimacs) report: pre- implant characteristics and outcomes. Ann Thorac Surg 2019;107:993–1004.
Page 12